The success of Ingenuity, otherwise known as the “Mars helicopter,” has united the world in celebration of a unique event in the history of aviation: the first powered flight by an aircraft on another world. Even the staid International Civil Aeronautics Organization got in on the act, designating the bare patch of Martian soil where the historic series of flights took place with its own three-letter airport code: IGY. Elon Musk has yet to announce when regular passenger service might begin, but at least now you will know what code to look for on the departure board.
It’s a heady moment in the history of flight, but for readers of Model Airplane News, there was something distinctly familiar about the entire episode. Strip away the exotic location and adaptations to fly in the rarefied and frigid atmosphere of Mars, and you are a left with a perfectly familiar model aircraft—and not an especially sophisticated one, at that.
Take it for a Spin
To begin with, Ingenuity’s coaxial propeller configuration is well known to modelers from low-cost helicopter models widely sold on Amazon for less than $50 by brands like Syma and Cheerwing. These are great aircraft for beginning pilots because they are inherently stable. Also, they can carry a greater payload than other types of rotorcraft with a comparable power source. Both traits are also much in demand on the red planet.
In addition, because the blades rotate in opposite directions, they cancel out the torque effects of one another on the airframe, holding the aircraft steady in its yaw axis. That means, unlike a conventional “single-rotor” helicopter, there is no need for a tail rotor—also called an anti-torque rotor.
Another benefit of the coaxial design is that it eliminates the problem of dissymmetry of lift. A helicopter’s blades are airfoils, exactly like the wings of an airplane. As the rotor passes through the air, it creates low pressure above each blade and high pressure below and the difference between the two generates lift. However, when a helicopter is in forward flight, the lift varies between the two sides of the aircraft.
The reason is that the blade moving in the same direction as the helicopter, referred to as the “advancing blade,” benefits from the additional airspeed generated by the movement of the helicopter itself—known as the “relative wind” to aeronautical engineering types. Greater airspeed equals greater lift. However, the “retreating blade” generates correspondingly less lift because it deducts, rather than adds, the relative wind to its effective airspeed, making the airfoil less efficient.
Because of its counter-rotating blades, a coaxial helicopter like Ingenuity does not suffer the effects of this dissymmetry of lift, contributing to its overall stability.
Another advantage of the coaxial helicopter design is that it occupies less physical space than a conventional helicopter, which is why crewed versions have been designed for deployment from naval vessels where deck space is at a premium. Multirotors, the most popular format for sUAS on Earth, also take up more space than a comparable coaxial platform. When you’re designing a rotorcraft to travel across 300 million miles of interplanetary space strapped to the belly of a rover, minimizing its overall size is crucial.
Of course, the atmosphere on Mars is only one percent as dense as our own, meaning the rotors must be larger and spin faster than they would here on Earth. However, their performance is hardly extraordinary by the standards of an ordinary model helicopter. The mainstream press has reported with seeming awe that Ingenuity’s rotors must turn at 2,500 rpm, compared with 500 rpm for a crewed helicopter. However, 2,500 rpm this is about on par with a typical RC helicopter.
(Really, Really) Remote Control
Both Ingenuity and a typical model aircraft are undeniably remotely controlled—there isn’t a pilot on either one—and the underlying technology that each one uses for command and control is basically the same: radio transmissions. Like a drone, Ingenuity maintains a two-way channel of communications with its Earthbound operators: receiving control inputs and sending back images and telemetry. However, as you might expect, there are some significant differences when it comes to the details.
Today, most model aircraft are controlled using a spread-spectrum signal at 2.4 GHz. This provides an extremely fast and robust connection between controller and aircraft—less than 25 milliseconds. Furthermore, by constantly leaping from one frequency to the next within the 2.4 GHz band, these systems deliver a high degree of reliability, despite all the other gadgets using the same frequencies: WiFi routers, cordless telephones, Bluetooth accessories, baby monitors and garage door openers, just to name a few.
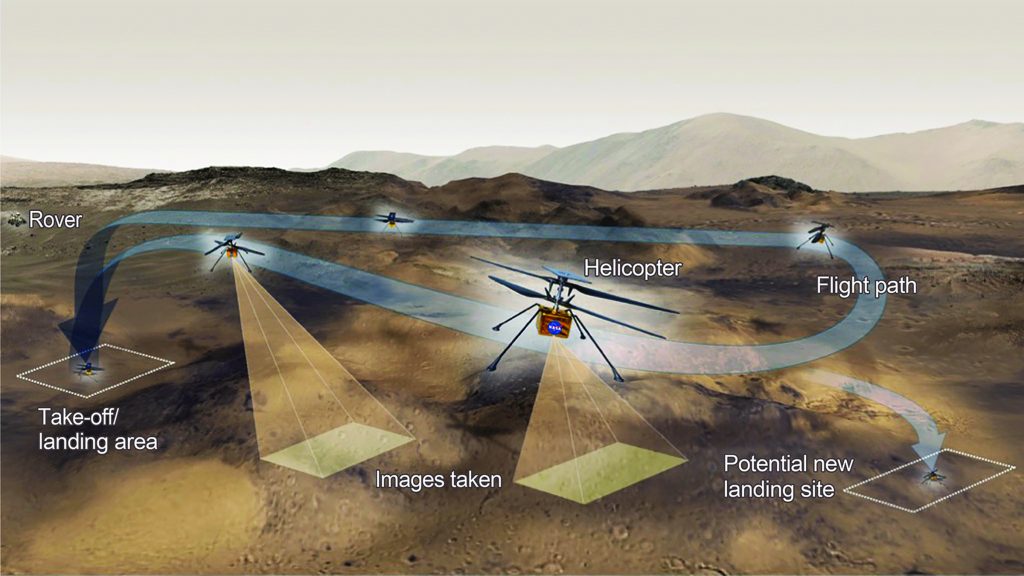
This diagram reveals the basic concept of operations (conops) that Ingenuity has been able to fulfill after its initial test flights demonstrating it was functioning as intended in the desolate Martian environment.
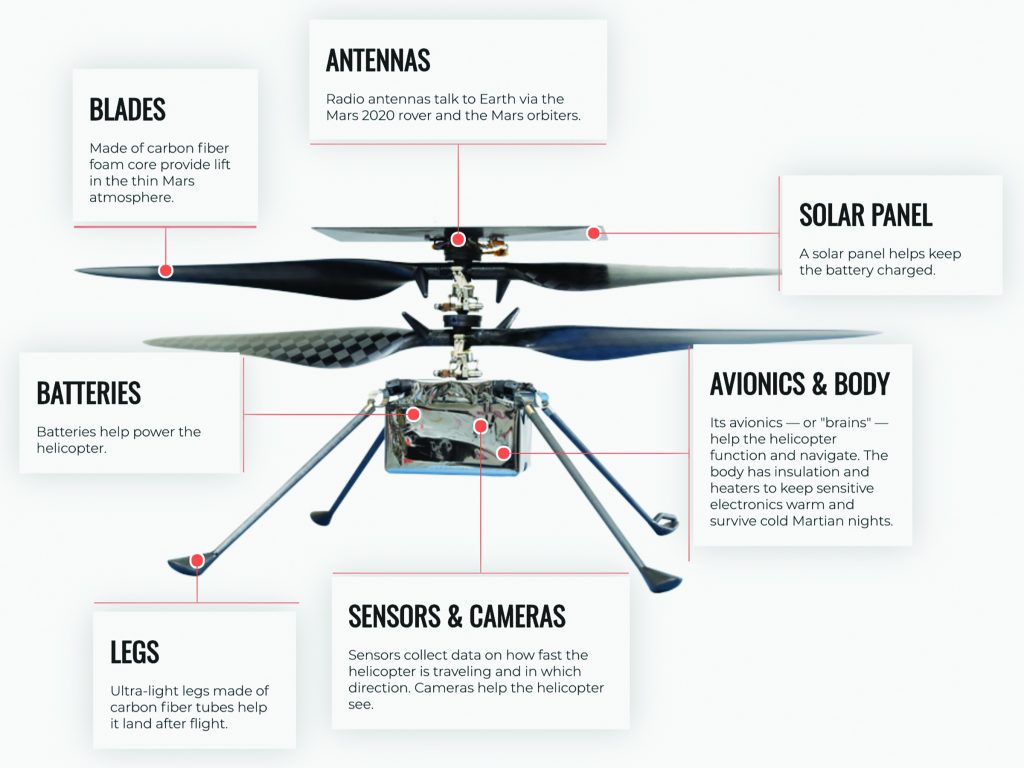
While costing $85 million and designed to fly above the surface of Mars, the Ingenuity helicopter shares many elements in common with the drones and model aircraft flown every day on Earth.
On drones, video and telemetry are typically also transmitted at 2.4 GHz, and they are slower by comparison—between 150 to 250 milliseconds, except for drones built specifically for first-person view (FPV) racing and freestyling. These intervals of time are literally the blink of an eye, so nearly imperceptible by human beings.
With Ingenuity, the same process takes rather more time, mostly due to the fundamental constraints of the universe as described by Albert Einstein a century ago. Radio waves travel through the vacuum of space at the speed of light: 186,000 miles per second. While that is ferociously fast compared to your morning commute, it’s well short of instantaneous when the distances involved are on an interplanetary scale. Depending on the relative position of the two planets in their orbits, the delay can be between three and 20 minutes.
Also, compared to a model airplane, the signal takes a rather more circuitous route. When Ingenuity has telemetry or images to share with its controllers on Earth, it begins by transmitting them via a short-range radio link to the Perseverance rover nearby. Then, Perseverance establishes a radio connection with the Mars Relay Network—a constellation of five satellites in orbit around the red planet, including NASA’s Mars Reconnaissance Orbiter (MRO), Mars Atmospheric and Volatile EvolutioN (MAVEN), Mars Odyssey, and the European Space Agency’s (ESA’s) Mars Express and Trace Gas Orbiter.
Unlike communications satellites around Earth, these are not located in geostationary orbit, so one must be above the horizon at Perseverance’s location before the rover can begin its upload, which only occurs at about half the speed of a household broadband connection. Then, the long journey to Earth begins.
Up to 20 minutes later, the signal is picked up by NASA’s Deep Space Network (DSN), an array of radio observatories with 230-foot dishes placed equidistant around the globe to ensure continuous contact with Perseverance and other deep-space missions. These are in the Mohave desert, outside of Madrid, Spain, and near Canberra, Australia. From there, it is sent to mission control at the Jet Propulsion Laboratory (JPL) in Pasadena, California.
So, this operation clearly does not occur within visual line of sight (VLOS), as required by the Academy of Model Aeronautics safety code. However, thus far the FAA has received no complaints.
Testing on Earth to Fly on Mars
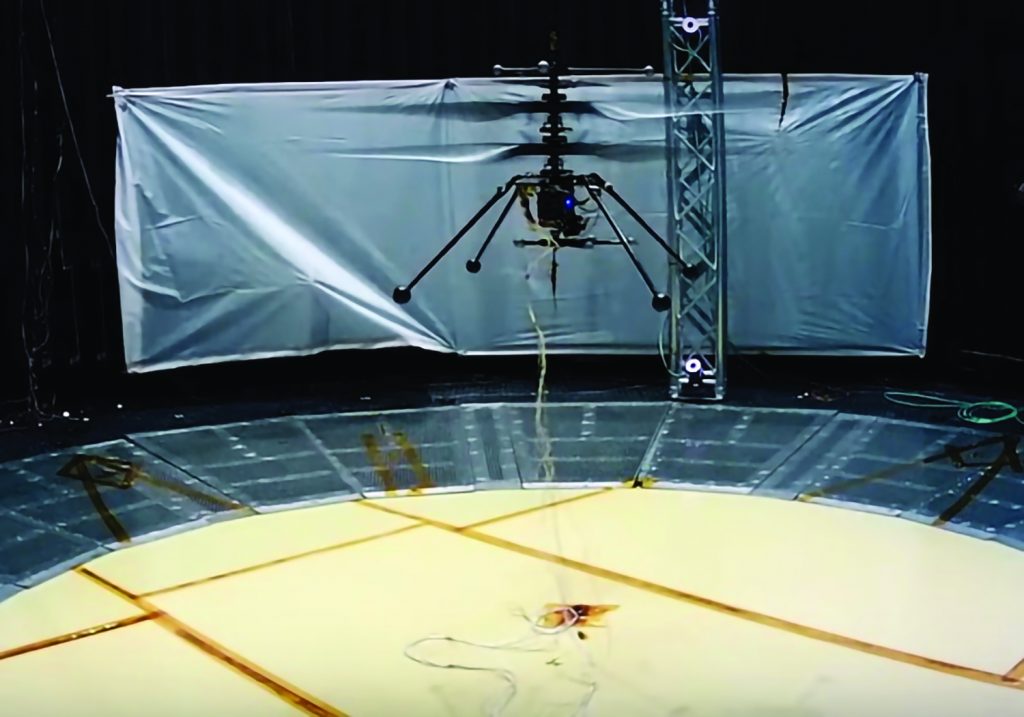
Inside the space simulator at the Jet Propulsion Laboratory in Pasadena, California, an Ingenuity prototype takes flight in a rarefied atmosphere to duplicate conditions on Mars.
Prior to landing on Mars, Ingenuity’s environmental tests were performed through modeling and computer simulations. Afterwards, the Jet Propulsion Laboratory’s Space Simulator—an 80-foot tall, 25-feet in diameter vacuum chamber was used to mimic the atmosphere on Mars. To see if Ingenuity could survive in certain weather conditions, engineers created wind tunnels and performed tests at predicted Martian temperatures. Then to mimic Mars’ gravity—which is one-third that of Earth—NASA used a high-tech fishing reel mounted like a pulley to offload the difference.
Yet, due to the tiny size of the Space Simulator, Ingenuity was not able to move more than 1.6 feet. This posed some unknowns; for instance, would the cameras and sensors be able to track the ground at higher speeds? Could dust interfere with camera performance? Will the algorithm for the camera run for long distances?
Ingenuity took 8 years to go from concept sketch to a fully engineered and operational reality. However, after only 27 sols and four successful flights, NASA was able to gain invaluable in-flight data that can be compared to Earth simulations, modeling, and tests. And with new data showing Ingenuity’s operating capabilities exceeding the original design limitations, it is safe to say that NASA engineers built a highly capable aircraft that is set to provide useful engineering data for missions to come.
Where am I?
Actually flying on Mars would be an unfamiliar experience for a model airplane pilot. Owing to the communications delay, each flight must be preprogrammed by controllers on Earth, transmitted to Ingenuity and then executed autonomously. This is nearly identical to the way a drone pilot might established a series of waypoints to map a quarry, for example, then press the “launch” button and monitor the aircraft’s progress without intervening directly unless emerging circumstances require it. Of course, when your aircraft is 34 million miles away and you won’t even know for sure that it has taken off before it lands, direct intervention isn’t really an option.
While performing autonomous operations on Earth, professional remote pilots and their aircraft depend almost entirely on two resources that are absent on Mars: GPS satellites and a planetary magnetic field. To overcome these issues, Ingenuity relies on versions of systems used on board drones every day here on Earth, adapted for the rigors and environmental conditions on the red planet.
The first among these is the black-and-white navigation camera mounted on Ingenuity’s belly. Capturing a meager 640 by 480 pixels 10 times a second, it functions in a manner identical to the optical flow cameras installed on nearly all commercial drones these days: by identifying distinctive features and tracking their changing position within consecutive images, it is possible to determine the direction and speed of the aircraft’s movement over the terrain—making a substantial contribution to its stability.
Also mounted on the underside of Ingenuity is a Garmin LIDAR Lite V3, functioning as a laser altimeter. Again, this is the exact same approach that drones use here on Earth, albeit using ultrasound instead of a laser beam. Flip over almost any drone made today and you’ll find what look like two tiny speakers—and that’s basically what they are. These emit a silent ultrasonic pulse and then listen for the echo after it strikes the ground below. Divide the time that takes in half and multiply it by the speed of sound, and you get a good estimate of the aircraft’s altitude. Horizon Hobby uses the same technology to power the Landing Assist Sensor (LAS) available for its popular Carbon Cub S2 1.3m trainer.
There are other similarities, as well. Ingenuity incorporates a cell-phone grade Bosch BMI-160 inertial measurement unit (IMU). Nearly identical hardware is incorporated in many current model airplanes. Using micro electromechanical systems (MEMS) to integrate three-axis gyroscopes and accelerometers into a solid-state circuit board, an IMU measures two critical variables aircraft performance: what is the relationship between the aircraft and the horizon, as defined in the pitch and roll axes; and how quickly is it changing?
This data is then fed into the aircraft flight control system (FCS)—a Qualcomm Snapdragon 801 microprocessor in the case of Ingenuity— =which uses it to make hundreds of changes per second to the performance of the aircraft to maintain stability. Even if a human pilot is providing real-time input, this sort of internal feedback loop is used to provide advanced features like Horizon Hobby’s Sensor Assisted Flight Envelope (SAFE) system, which limits aircraft pitch and roll to help new pilots who are learning how to fly.
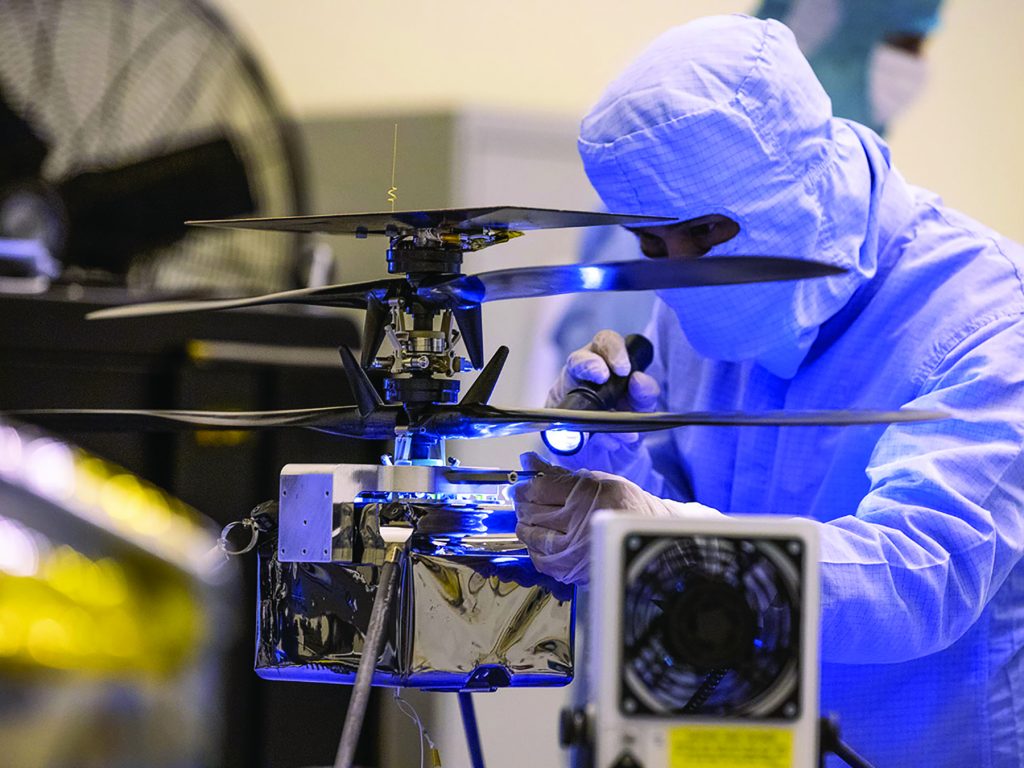
Taking meticulous care to avoid any biological contamination that could inadvertently be carried to Mars on board Ingenuity, a technician at the Jet Propulsion Laboratory inspects the helicopter prior to its transfer to Perseverance.
Cold Comfort
Not only would the batteries that power Ingenuity be familiar to model airplane pilots, but an especially capable hobbyist might also be able to rig them to power a small aircraft here on Earth. The Mars helicopter uses six Sony lithium-ion batteries with a total capacity of 2,000mAH. That’s about the same as the battery used on a typical 1.5 meter fixed-wing foamie, providing 12 to 15 minutes of flight time, depending on how aggressive you are with the throttle.
Once again, despite the overall similarity, there are some key differences between how these power systems work on Earth and Mars. To begin with, Ingenuity typically only flies for 90 seconds, with a maximum endurance of about two minutes. Also, there are no wall outlets on Mars, so Ingenuity must rely on a solar panel fixed atop its rotors to recharge its batteries.
However, the most crucial difference in this regard is the bitter cold of the red planet, especially at night, when temperatures can dip as low as 130 degrees Fahrenheit. If you have ever tried to fly a model aircraft on an especially cold day here on Earth, then no doubt you have noticed that you get less performance out of your batteries. The reason for this is that as the temperature drops, the internal resistance within LiPo batteries increase.
Effectively, the total amount of electrical energy stored within the battery remains unchanged; however, some proportion of that energy must be spent simply to get the electrons flowing to the aircraft systems, with the practical effect that less energy is available to fly.
For professionals who must operate small drones in extremely cold environments, like the arctic, manufacturers have developed systems to help keep batteries warm. For example, the DJI Mavic Enterprise series uses intelligent batteries that are programmed so that when their internal temperature drops to between 14- and 42.8-degrees Fahrenheit and the remaining battery power is above 50 percent, self-heating will automatically begin. Once the battery is above 46.4 degrees Fahrenheit, the battery will enter a heat retention mode and remain in that condition for 20 minutes.
Ingenuity uses a similar system. First, it is insulated, which reduces the loss of heat into the environment. Second, just like intelligent drone batteries here on Earth, the Mars helicopter discharges its batteries during periods of extreme cold to keep themselves—and the aircraft’s electronics—warm.
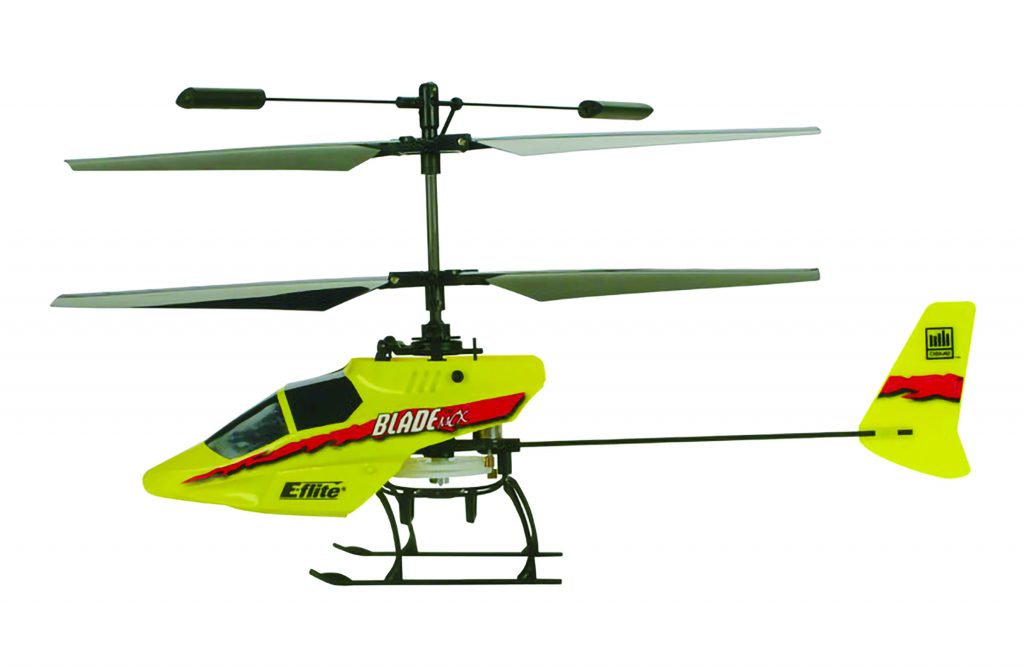
Coaxial helicopters, like this Blade mCX, are a good choice for first time pilots because the configuration is inherently stable and energy efficient, which also make it a good choice for the first flying machine on another planet. (Image courtesy Horizon Hobby)
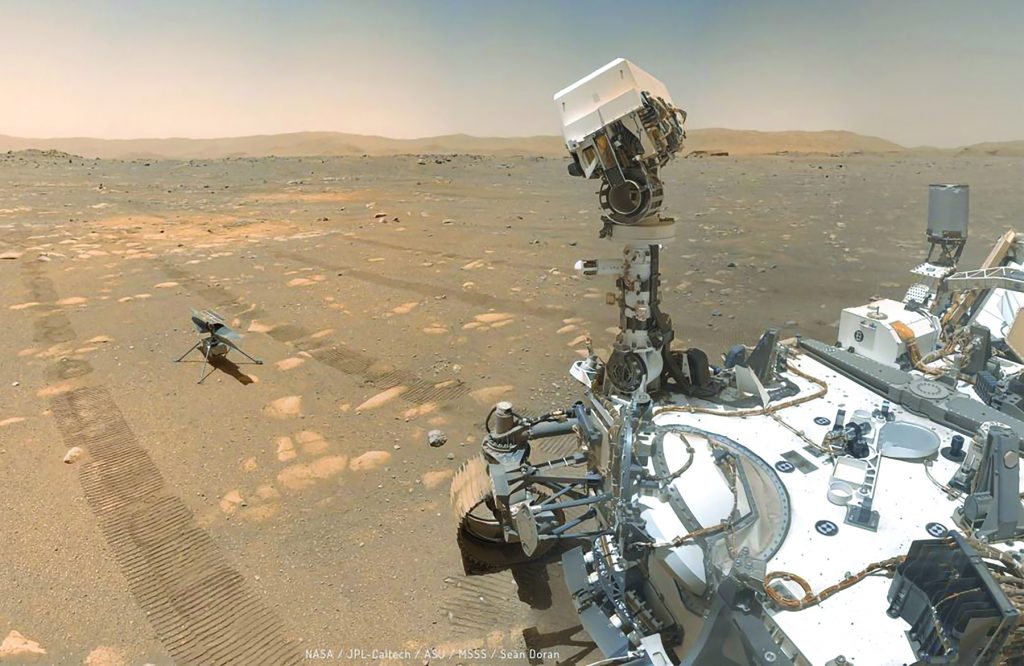
Like a proud parent, the Perseverance rover keeps watch over Ingenuity, standing alone on the Martian surface prior to its first test flight.
The View from Above
The purpose of flying drones—on Earth, anyway—is typically to position a sensor in three-dimensional space: to capture dramatic aerial video or photographs, to create a detailed map of a specific site, or to search for a subject lost in the wilderness using thermal imaging. However, the purpose of Ingenuity recalls an earlier era in model aviation: simply to demonstrate that remotely controlled flight is even possible.
For aeromodelers, this reflects the period during the 1930s when pioneers such as Chet Lanzo and twin brothers William and Walter Good began to experiment with converting gas-powered free-flight models to radio control using rubber-band powered escapements. For those forerunners of the community that exists today, the goal was to simply prove that they could control a flying machine remotely.
Likewise, simply lifting itself above the Martian surface for a few precious seconds is Ingenuity’s overriding goal. Therefore, the most common payload carried on drones today—a visible-light camera—is essentially an afterthought, largely unrelated to the success of the mission.
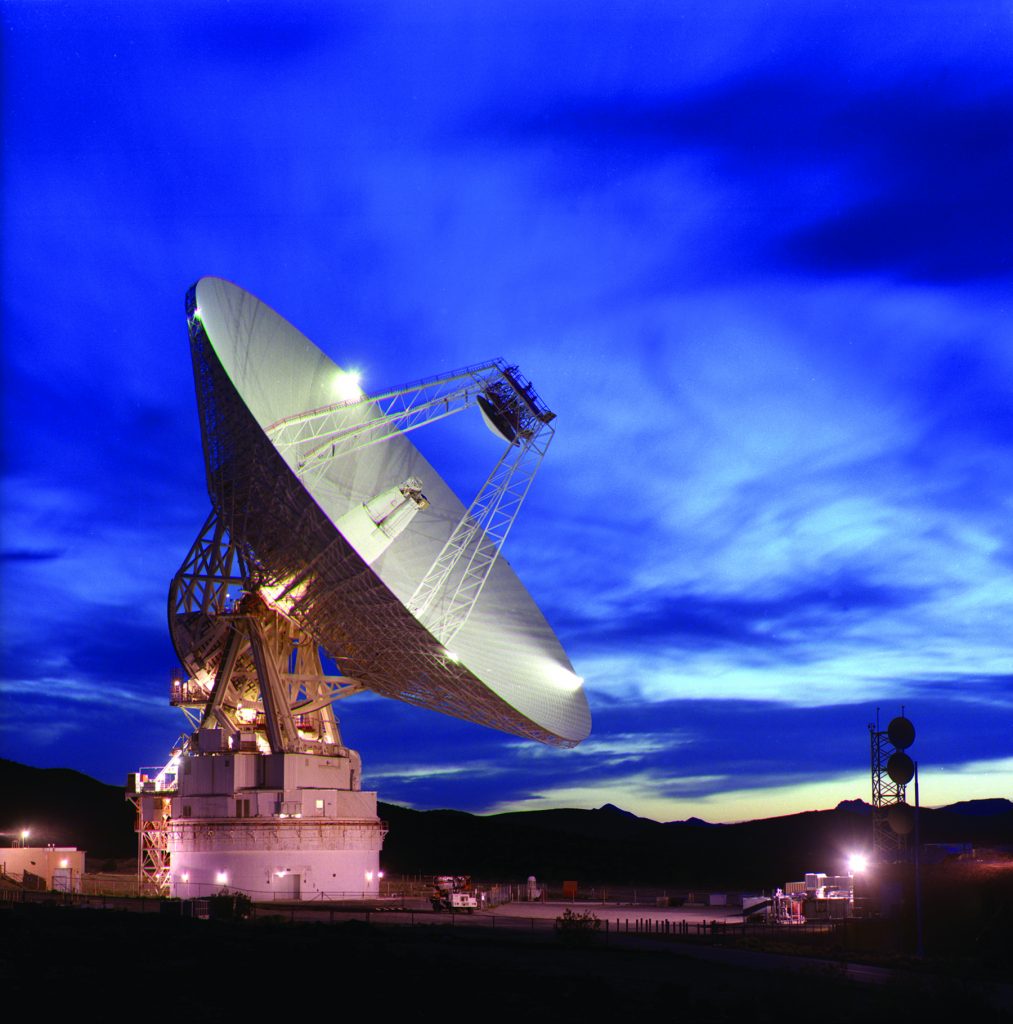
Located in the Mohave Desert, the Goldstone Deep Space Communications Complex is home to this 230-foot dish capable of transmitting and receiving signals from Perseverance and other deep space missions. Together with similar facilities in Spain and Australia, these comprise NASA’s Deep Space Network (DSN).
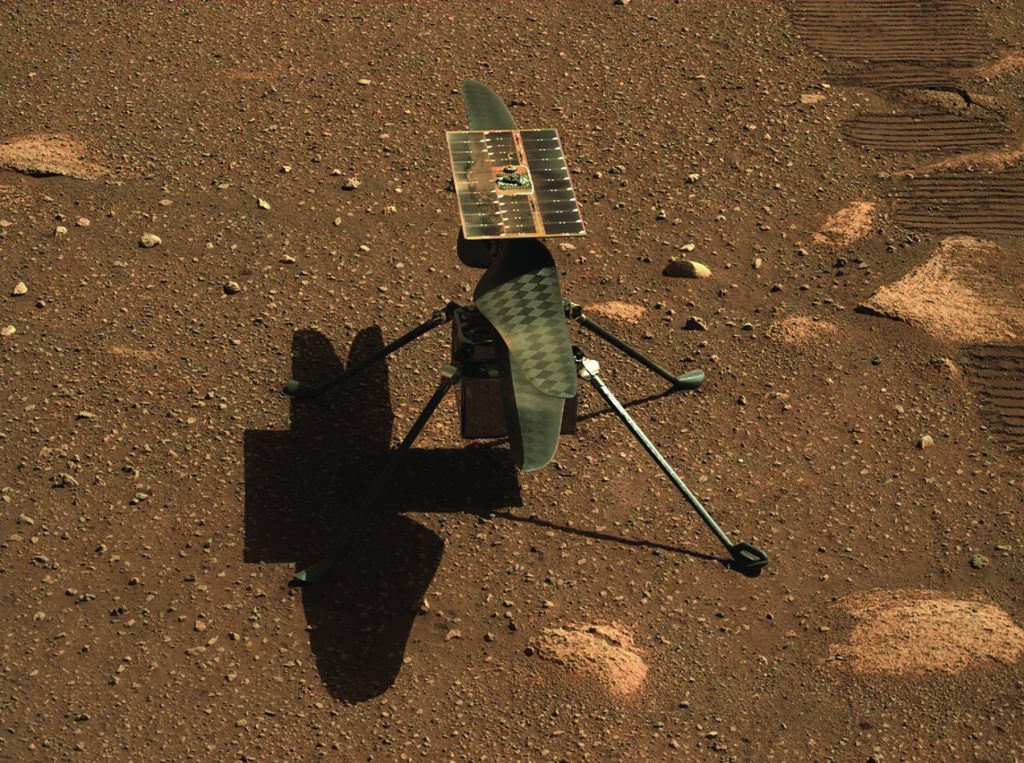
With Perseverance’s tracks still visible in the background, Ingenuity recharges is on-board lithium-ion batteries using the solar panel mounted above its propeller blades.
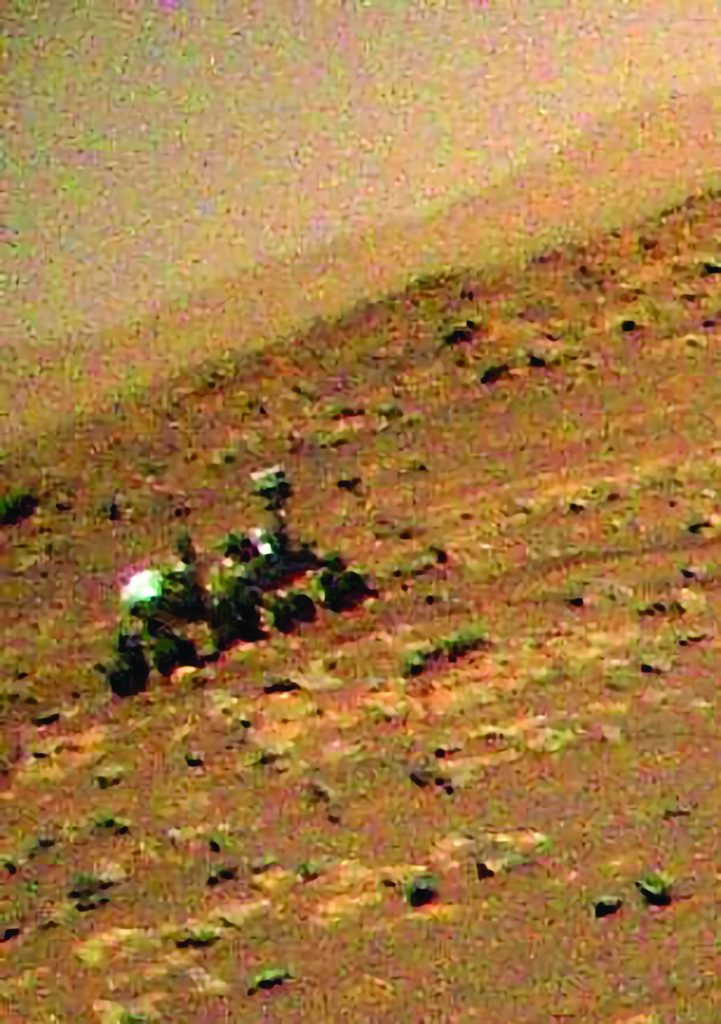
Without the benefit of a gimbal, the color camera mounted on the front of Ingenuity reflects the pitch and roll of the aircraft in the images it captures. In this instance, the helicopter caught a glimpse of Perseverance, parked at a safe distance during flight testing.
That is clear when you compare the color camera that NASA sent to Mars on board Ingenuity with what you will find on an average consumer drone. First, it is hard-mounted to the aircraft, with no gimbal to stabilize the image. For that reason, the pitch and roll of the aircraft is reflected in the jarring angle of the horizon in the images sent back by the Mars helicopter. Also, with a resolution of 4,208 by 3,120 pixels, the images it captures are approximately 12 megapixels, as compared with the 20-megapixel camera incorporated into the recently released DJI Air 2S.
So, by some measures, Ingenuity is quite primitive when compared with the drones being flown on Earth every day. However, in the history of model aeronautics, we can see the inexorable path forward for the future of aviation on worlds other than our own. This early experiment will rapidly yield to far more robust and capable platforms that would astonish the lonely pioneers who first accomplished what few dreamed was even possible.
The development of RC is an amazing story, and there are those among us who lived through it. Yet, these are small stories, known only by a few. With the advent of uncrewed aircraft on other planets, all of humanity is embarking on this extraordinary journey together.
About the Authors
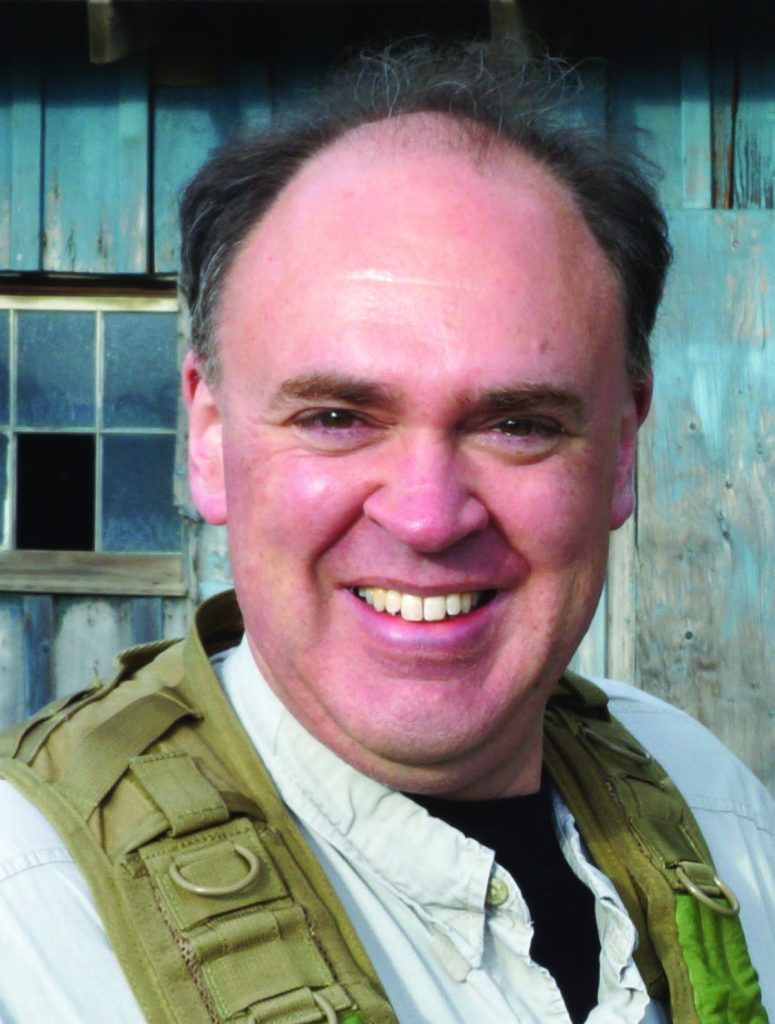
Patrick Sherman
Patrick Sherman is a pioneer in the drone industry and an Instructor of the Practice at the Embry-Riddle Aeronautical University Worldwide Campus Department of Flight. A popular writer, speaker and educator across the industry, he is the founder of the Roswell Flight Test Crew. Patrick is a Trusted Operator Program (TOP) Level 3 Remote Pilot Instructor and a DronePro with the Federal Aviation Administration Safety Team (FAAST). He has been recognized as the Drone Instructor of the Year by the Association for Unmanned Vehicle Systems International (AUVSI).
Tunesha Griffith
Tunesha Griffith is an Unmanned Systems Applications major at Embry-Riddle Aeronautical University (ERAU), currently living in Wiesbaden, Germany. Previously, she worked in the information technology and video game industry in community development, event promotions, and social responsibility initiatives. At ERAU, Tunesha is pursuing her study of autonomous transportation systems, unmanned space vehicles, and smart robots. Her goal is to work in a field that allows her to combine her love for helping others with advances in unmanned systems.
ERAU
Embry-Riddle Aeronautical University affords students the opportunity to work with world-renown experts, both in class and in professional partnerships.